Genetic determinants of periosteum-mediated craniofacial bone regeneration: a systematic review
Article information
Abstract
Background
Periosteum-mediated bone regeneration (PMBR) is a recognized method for mandibular reconstruction. Despite its unpredictable nature and the limited degree to which it is understood, it does not share the concerns of developmental changes to donor and recipient tissues that other treatment options do. The definitive role of the periosteum in bone regeneration in any mammal remains largely unexplored. The purpose of this study was to identify the genetic determinants of PMBR in mammals through a systematic review.
Methods
Our search methodology was designed in accordance with the PRISMA (Preferred Reporting Items for Systematic Review and Meta-Analysis) guidelines. We conducted a quality assessment of each publication, and evaluated the differences in gene expression between days 7 and 15.
Results
A total of four studies satisfied the inclusion criteria. The subjects and tissues examined in these studies were Wistar rat calvaria in two studies, mini-pigs in one study, and calves and mice in one study. Three out of the four studies achieved the necessary quality score of ≥ 3. Gene expression analysis showed increased activity of genes responsible for angiogenesis, cytokine activities, and immune-inflammatory responses on day 7. Additionally, genes related to skeletal development and signaling pathways were upregulated on day 15.
Conclusions
The results suggest that skeletal morphogenesis is regulated by genes associated with skeletal development, and the gene expression patterns of PMBR may be characterized by specific pathways.
INTRODUCTION
Reconstructing the mandible in young patients is considered uncommon and complex [1,2]. To date, approximately 30 cases of pediatric mandibular reconstruction have been documented in the English literature. Of these, 13 involved free flap reconstructions, 11 used non-vascularized reconstructions, and seven employed both techniques [1-30]. The intricacy of this procedure arises from the need for a thorough understanding of the developmental changes in bone and soft tissue at both the donor and recipient sites [26]. The choice of treatment for a mandibular defect, a crucial part of managing jaw tumors, varies based on the geographical and developmental context. Western centers often opt for primary or secondary reconstruction with vascularized osseous flaps and distraction osteogenesis. In contrast, developing healthcare systems like ours in Nigeria frequently use non-vascularized bone grafts, capitalizing on the high incidence of benign mandibular lesions in children [26-28,31]. A third treatment option, periosteum-mediated bone regeneration (PMBR) of the mandible, is commonly used in developing healthcare systems and is gradually gaining acceptance at Western centers [32,33]. To date, 32 case series involving 63 cases have been reported [32-63], with 44% (28/63) of these cases originating from Africa [33,34,41,50,52,54,55,57]. This trend may be due to limited facilities that hinder immediate or vascularized reconstruction. Despite the limited understanding and unpredictability of PMBR, there are no concerns about changes in donor and recipient tissues during growth and development.
In our previous clinical work on PMBR of the mandible in humans [33], we, like others, described it as an unexpected clinical phenomenon following periosteum-preserved mandibular resection by sub-periosteal dissection (Fig. 1A). This process is characterized by bone formation induced by the periosteum in an iatrogenic mandibular defect, provided three conditions are met: an intact periosteum, a mandibular segment as an inducer agent, and a young patient [32,39,64]. While these three conditions are generally required for PMBR, they are not absolute. There have been reports of the entire mandible regenerating [52] and adult periosteum undergoing bone regeneration, despite the preference to use PMBR with young periosteum [37,39]. This suggests that young periosteum may retain its osteocompetency despite increasing age [33]. PMBR is only indicated for benign lesions, as the periosteum needs to be preserved. Regarding its protocol, we reported that the regenerative potential of the mandibular periosteum begins as early as 7 to 10 days and can regenerate up to a bimandibular regenerative span of approximately 8 cm in length. We also noted that PMBR for the mandible can be classified as “complete” (i.e., regenerated bone occupying > 50% of the radiographic defect) or “incomplete” (i.e., irregular in outline and occupying < 50% of the radiographic defect) (Fig. 1B) [33].
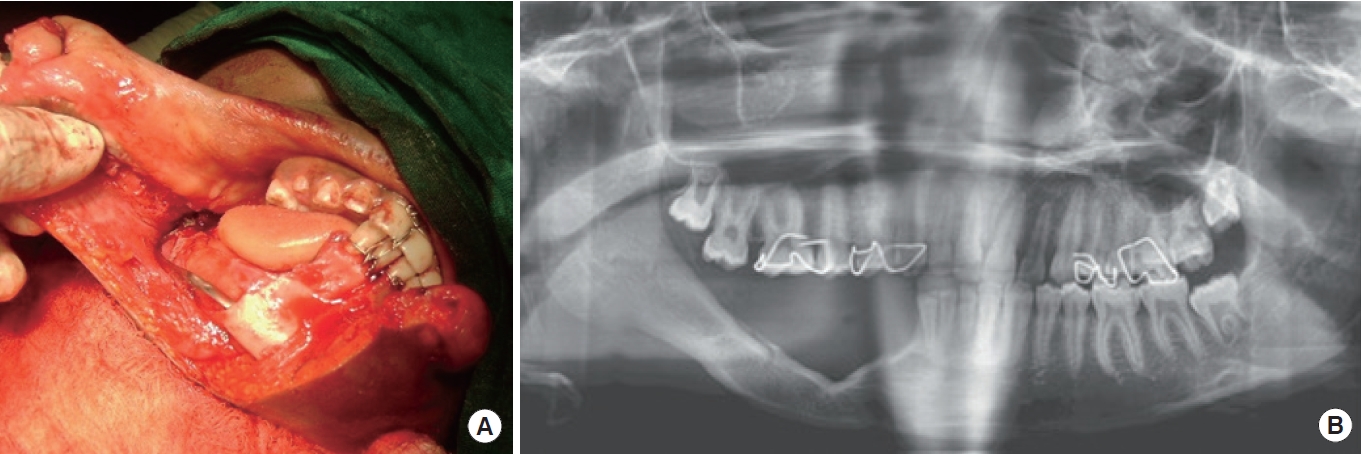
A case involving sub-periosteal dissection with arch bar placement. (A) A 10-year-old boy with right mandibular unicystic ameloblastoma (canine to ramus). (B) At 11 months postoperative, with complete periosteum-mediated bone regeneration.
While research on bone repair and regeneration is ongoing, the definitive role of the periosteum and the molecular pathways that regulate bone regeneration in mammals remain largely unknown [65]. Genetic determinants, as revealed through expression analysis, involve gene transcription and translation to functional gene products (RNA and protein) (Fig. 2). This is considered an accurate method for investigating genetic associations, as it provides insight into normal cellular processes [66]. Gene expression analysis can be broadly divided into RNA expression by transcription, protein expression by translation, and post-translational modification. However, RNA and protein expression levels are most commonly used for analysis. The techniques used to analyze gene expression include DNA arrays and real-time polymerase chain reaction (RT-PCR) for RNA expression, and Western blotting and gel electrophoresis for protein expression analyses [66,67]. The aim of this study was to conduct a systematic review of the genetic determinants of PMBR in mammals.
METHODS
Search strategy
Our search methodology was designed in accordance with the PRISMA (Preferred Reporting Items for Systematic Review and Meta-Analysis) guidelines [68]. We used a Cochrane-style approach, employing MeSH terms and keywords related to periosteum, craniofacial and/or bone regeneration, as well as associated gene expression studies and techniques such as DNA array and RT-PCR. The initial search was conducted using the following tools: PubMed, Ovid Medline, and Web of Science.
Eligibility criteria
Only English-language publications were reviewed, and the selected studies included cross-sectional studies, case-control studies, and controlled clinical trials. The titles and abstracts of these publications were reviewed to identify suitable articles, and the selected manuscripts were then proofread. Additional publications were retrieved and reviewed from the reference list of this initial search to identify potential papers that met the study’s criteria. All studies were required to use proprietary named kits for gene expression studies, in accordance with the manufacturer’s protocol. Articles that were purely clinical, related to pathology, conference proceedings, or only abstracts were excluded.
Quality appraisal
The quality of each publication was assessed using a modified version of a pre-existing tool, Strengthening the Reporting of Genetic Association studies (STREGA) [69]. This involved evaluating each article for the following elements: characterization of periosteum-mediated craniofacial bone regeneration, description of the case and control screening population, inclusion of gene or variant ID (e.g., National Center for Biotechnology Information rs identification, evaluation of gene function or Gene Ontology identifiers), and measurement of genetic associations. Additionally, the difference in gene expression between 7 and 15 days was reviewed. This time frame was chosen based on reports that bone regeneration can begin as early as 7 to 10 days [33].
Data extraction and analysis
All results were compiled into a data form for tabulation. We used the SPSS 27 software package (IBM Corp.) for statistical analysis. We recorded the sample size, age range, and mean or median of subjects with paired sample data during the expression analysis to provide descriptive statistical information. When a microarray was used to analyze differential gene expression, we applied a fold change threshold in accordance with the manufacturer’s protocol to determine the significance of gene expression.
RESULTS
The electronic search yielded 69 citations. However, 57 of these publications were discarded due to their lack of relevance to the search objectives. Specifically, 38 were descriptive studies, nine were conference proceedings, eight were observational studies, and two were duplicate studies. This left 12 publications for a full-text review. After a more detailed review, an additional eight studies were eliminated because they were purely clinical (observational) and had no correlation with gene expression. Consequently, only four studies [70-73] met the inclusion criteria (Fig. 3).
Three out of the four studies had a modified STREGA score of ≥ 3 (Table 1). The study subjects and tissues were Wistar rat calvaria in two studies, mini-pigs in one study, and calves and mice in one study. The techniques used to examine gene expression included microarrays, RT-PCR, and transcriptomics, with results assessed on days 7 and 15. In summary, the results indicated an increase in the activity of genes responsible for angiogenesis, cytokine activities, immune-inflammatory responses, and skeletal development. Genes associated with cytokine and immune-inflammatory responses, such as cytokines (interleukin-1β and interleukin-6), cathepsin K, and receptor activator of nuclear factor kappa-B ligand, were primarily implicated on day 7. In contrast, osteoblast-specific genes, including those coding for runt-related transcription factor 2, collagen type 1, osteonectin, and osteocalcin/osteopontin, were primarily implicated on day 15 (Table 2). Additionally, several biological signaling pathways, such as bone morphogenetic protein (BMP)-2, hedgehog, PDGF, Notch, and Wnt, were also implicated on day 15 (Table 3).
DISCUSSION
The bones in the craniofacial and appendicular regions develop differently. Specifically, the former originates from ectodermal neural crest cells of the closing neural tubes, which gives its periosteum and ossification patterns distinct characteristics compared to appendicular bones [74-76]. Craniofacial bone matures through an intramembranous process, characterized by various mesenchymal cells differentiating into bone-depositing osteoblasts or ossification centers, and eventually forming compact bone [77]. In contrast, appendicular bone develops through endochondral ossification, characterized by cell differentiation into chondrocyte-synthesized collagen, which matures through proliferation and then templates the shape of the future bone [78,79]. Despite these differences, intramembranous and endochondral bone developments share similar regulatory mechanisms [77,80].
Bone repair or regeneration is generally believed to be driven by the periosteum, but the precise role of the periosteum in bone regeneration remains unclear [77,78]. It consists of four successive phases: an initial inflammatory response and recruitment of osteo-progenitor cells, formation of a cartilaginous template, replacement of the template with immature (spongy) bone, and finally remodeling into mature bone. The periosteum is the primary driver throughout all four phases [80]. In the author’s opinion, an improved understanding of the periosteum’s role could provide a clear solution for bone repair or reconstruction, offering benefits such as low cost and reduced morbidity from a non-existent donor site. However, its unpredictability and lack of consensus among clinicians remain significant drawbacks. While few genetic association studies on PMBR have been conducted in humans, some have been carried out in animal models, which was the focus of this study.
The limited number of studies that met this study’s inclusion criteria highlights the scarcity of publications on this topic, even in animals. Additional searches revealed no reports on the underlying molecular mechanism of the periosteum in bone regeneration. One of the four studies that met the inclusion criteria was deemed to be below the required quality score due to a lack of Gene Ontology identifiers [73]. This same report also used RT-PCR, unlike the other three studies that used microarrays. The absence of Gene Ontology identifiers prevented specific gene expression interpretation, which would have facilitated the comparison of both techniques.
According to this review, skeleto-developmental and immune-inflammatory responses were the major events implicated in bone regeneration. The reports suggest that the inflammatoryimmune response was unique and different from that of typical soft tissue wound healing [81-83]. In addition to these two mechanisms, angiogenesis and neurogenesis also occurred. While new vessel formation is a well-recognized requirement for bone regeneration, neurogenesis was somewhat surprising. However, there have been reports suggesting that new nerve fiber formation promotes osteogenesis [84-86]. Furthermore, references to gene expression in PMBR were sought between 7 and 15 days because PMBR manifests clinically as early as 7 to 10 days. The upregulation of inflammatory and immune response at 7 days was expected, and this supports the initial inflammatory phase of bone regeneration. However, the additional responses of the I-kB kinase/NF-kB signaling pathway were interesting, as this is a known pathway reported to induce inflammation-induced bone loss [87]. In week 2, the upregulated pathways (transforming growth factor [TGF]-beta/BMP, Wnt, and Notch) are known to upregulate skeletal developmental gene (SDG) expression. While these pathways are well-known skeletal development pathways, BMP signaling is the only pathway suspected to be associated with bone regeneration, and BMP2 is suggested to regulate PMBR through periosteum-based target cells [88,89]. Additionally, the Wnt signaling pathway was another upregulated response noted in week 2. However, unlike the Bmp target cells, the Wnt target cells reside in fractured bones, suggesting that the Wnt pathway might influence more of bone repair than regeneration, albeit through a different mechanism from the Bmp signaling [90,91].
While PMBR shows significant potential for bone reconstruction, particularly in the mandible, the impact on developmental growth following pediatric mandibular reconstruction remains uncertain and requires further research. This uncertainty arises from several factors. For instance, the condyle is a site of mandibular growth, and whether it is involved or preserved could influence jaw growth after PMBR. While it is known that factors such as the patient’s age can affect the rate of growth, other factors such as radiotherapy and chemotherapy are not a concern because PMBR is not recommended for malignant lesions [12,26,33].
In conclusion, this review aimed to harmonize various reports on the intricate processes of PMBR in the mandible, focusing on gene expressions and signaling pathways. The findings suggest that the gene expression patterns of PMBR may be characterized by skeletal morphogenesis, which is regulated by SDGs and pathways. Immune-inflammatory genes seem to be predominantly upregulated in the first week, while SDGs and signaling pathways are upregulated in the second week. One limitation of this study is that it only characterized events based on whether gene regulation is up or down between the first and second week. Furthermore, due to the tissue heterogeneity in the periosteum, it was impossible to attribute a specific phenotypic or molecular event to a particular cell type. This was due to the lack of histological analysis, which meant the source of these events could not be localized. Additionally, the analysis only considered actively expressed genes at the transcription level, which may not correspond to the protein coded for or expressed. Therefore, integrating the assays used in these reports with immunohistochemistry or proteomics may provide a more accurate depiction of bone regeneration events, and could be a focus for future studies. Lastly, factors such as the patient’s age at the time of reconstruction and condylar preservation should be carefully considered during PMBR to ensure optimal results.
Abbreviations
BMP
bone morphogenetic protein
PMBR
periosteum-mediated bone regeneration
RT-PCR
real-time polymerase chain reaction
SDG
skeletal developmental gene
STREGA
Strengthening the Reporting of Genetic Association studies
TGF
transforming growth factor
Notes
Conflict of interest
No potential conflict of interest relevant to this article was reported.
Funding
None.
Patient consent
The patient’s guardian provided written informed consent for the publication and the use of patient images.